
Expired activity
Please go to the PowerPak
homepage and select a course.
Nanomedicine: A Primer for Pharmacists
INTRODUCTION TO NANOTECHNOLOGY
Definition
Nanotechnology was first introduced as a concept by physicist Robert Feynman in 1959 during a talk he delivered at Caltech University. Since then, nanotechnology has markedly expanded into many fields, including physics, chemistry, biology, medicine, electronics, and information technology.1-4
While the National Institutes of Health (NIH) defines nanoparticles (NPs) as structures ranging from 1 to 100 nm in at least 1 dimension, the general term of nanotechnology refers to the engineering, control, and application of structures, materials, devices, and systems with properties and functions, in the nanoscale, specifically ranging from 1 to a few 100 nm.5-8
Most nanomaterials have special physical and chemical properties that differ from parent “bulk” materials, such as melting point, fluorescence, electrical conductivity, magnetic permeability, and chemical reactivity, which change as a nonlinear function of the size of the particle at this nanometer size range.9-12
Hence, this nanoscale science investigates unique phenomena to enable novel applications, where nanotechnology involves fabricating, imaging, measuring, modeling, and influencing matter at this length scale.1,13
Nanomedicine: An Offshoot of Nanotechnology
The advent of nanotechnology in the medical field, gave rise to the term “nanomedicine”, dating back to the 1990s. Nanomedicine can be broadly defined as the biomedical application of nanoscale materials for the diagnosis and therapy of diseases. Therefore, by extrapolating the official definition of nanotechnology, it was advised that for a nanoscale platform to be classified as a nanomedical product, the nanopharmaceutical product must meet 2 major criteria 2,14-17:
- Nanoengineering must play a major role in the manufacturing process, with specific control over the nanoscale dimensional size
- The nanomaterial used must be either essential for the therapeutic activity or must confer additional and unique properties to the active drug entity.2,14-17
Various nanostructures with diverse dimensions, forms, compositions, and functions have been implemented, and many nanomedicine products have been authorized by the United States (US) Food and Drug Administration (FDA) for clinical use over the past 3 decades.16,18,19
NANOMEDICINE: AN OVERVIEW
Typical/Conventional Drug Development
Nanotechnology plays an important role in the advancement of the field of medicine and drug delivery, due to the major limitations and problems that affected original therapeutic agents, and conventional formulations and delivery systems.20,21
In comparison to conventional drug formulation (including either freely soluble in solution or mixed within solid dosage form), the encapsulation or incorporation of drug molecules within nanocarriers (NCs) generally means that the physicochemical properties of the nanomedicine formulation become mostly governed by those of the NP delivery system. Hence, alterations and/or tuning of the NC properties can significantly improve the overall properties and characteristics of the whole nanomedicine formulation. 13,20,21
One of the significant problems of several conventional formulations is the difficulty in removing some of the residual components (eg, manufacturing additives and excipients) of such delivery systems, potentially leaving nonbiodegradable trace material within the patient’s body, which may cause toxicities or hypersensitivity reactions.3,21 In addition, most conventional drug formulations exhibit a high initial burst of drug release immediately following administration, especially for water-soluble compounds. Conversely, several active drug molecules for infectious diseases and many anticancer chemotherapeutic agents are hydrophobic, posing challenges for solubilization, and systemic administration via conventional formulation platforms, with marginal bioavailability.12 Moreover, while the hydrophobic nature of these drugs leads to easier absorption across cellular membranes, it is also responsible for aggregation and local embolism, leading to complications and adverse effects.20 Therefore, nanopharmaceuticals can offer promising solutions for the above-mentioned problems,21,22 as NC systems have several advantages, owing to their unique nanoscale properties.3, 20-22
Concept of Nanomedicine in Existence for Decades
Due to their unique features, NC systems for clinical therapeutic agents have the potential to revolutionize therapy via personalized medicine. Back in 1976, Gregoriadis described nanoformulation strategy as putting “old drugs in new clothing”, in reference to the liposomal drug NCs, as an archetypical nanolipid pharmaceutical platform.2,5,21
For many years now, it has been demonstrated that NC systems are much smaller than the basic material unit of conventionally formulated drugs. The incorporation of active drug substances within NC platforms protects it from the external environment while increasing the surface area. Nanosized drug formulations, in comparison with conventional micronized ones, almost always lead to an increased active concentration and improved systemic bioavailability. Consequently, drug nanovehicles help reduce the overall administered dose and potentially toxic adverse effects.21,22
Impact on Diagnostics, Imaging, and Medicines
To be eligible for preclinical and clinical applications, nanomedicine must be composed of materials that are biocompatible, nontoxic, and biodegradable.22 Moreover, they should be stable after administration and, in view of possible clinical translation, their preparation is easily upscaled for manufacturing with high control over their physicochemical properties.21
Since 1970, the FDA’s Center for Drug Evaluation and Research (CDER) has received more than 600 applications (investigational new drug [IND], new drug application [NDA], abbreviated new drug application [ANDAs]) for human drug products containing nanomaterials—half of which were submitted within the last 10 years (FIGURE 1).4,21 Most of the nanotherapeutic products currently approved by the FDA are meant for parenteral administration and only a few preparations have been designed for topical/localized application and even less for systemic oral administration (TABLE 1 lists some notable examples of the approved nanomedicines currently in clinical practice).4,14
FIGURE 1. Human Drug Product Submissions to FDA-CDER That Contain Nanomaterials (1970-2020)4,14 |
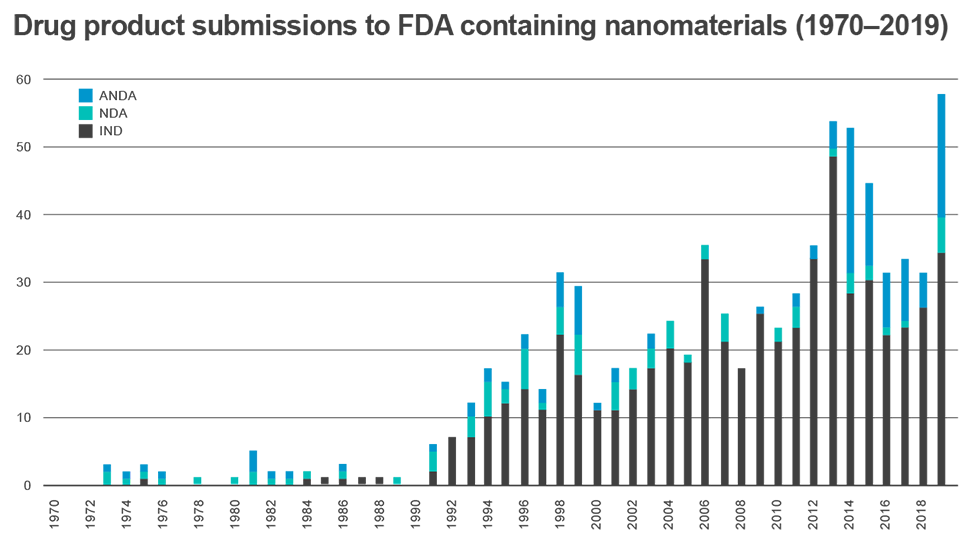 |
Abbreviations: ANDA, abbreviated new drug application; CDER, Center for Drug Evaluation and Research; FDA, Food and Drug Administration; IND, investigational new drug; NDA, new drug application. |
The first clinically approved nanomedicine was AmBisome in the 1990s (EU then US), a liposomal formulation of amphotericin B for the treatment of fungal infections.18 The advantage of amphotericin B encapsulation into high transition temperature phospholipids and cholesterol improved the drug’s clinical efficacy and reduced its overall systemic toxicity and reported adverse effects, in comparison to conventional amphotericin B IV solutions.2,23
Today, most nanomedicines on the market are indicated for cancer treatment, but other applications include mental illness, anemia, and inflammatory diseases such as rheumatoid arthritis, inflammatory bowel disease, asthma, multiple sclerosis, and diabetes (see TABLE 1 for examples).22,23 Over the past 3 decades, the application of nanomaterials in biomedical imaging as contrast agents for anatomical and functional visualization has become a growing focus of nanomedicine research and development. The utilization of nanomaterials as contrast agents enables time-resolved visualization of organ, tissue, and pathological structures inside the human body and helps clinicians to identify healthy from abnormal tissues, thus coming to a more accurate clinical diagnosis to recommend the most suitable treatment. Owing to their modular size, structure, and surface, nanomedicines can be custom nanoengineered with different contrast properties. The most common diagnostic imaging modalities for utilizing nanoscale contrast agents are computed tomography (CT); magnetic resonance imaging (MRI); imaging of radiotracer activity, such as positron emission tomography (PET) or single photon emission computed tomography (SPECT); fluorescence imaging; and photoacoustic imaging. For all these imaging techniques, modifiable nanomedicine contrast agents not only enable visualization of biological tissues’ structure and function, but these advanced imaging platforms can also be nanoengineered to specifically localize in target tissues and produce high contrast.1,4,13,14
TABLE 1. Important Examples of Nanomedicine Products in Current Clinical Practice14 |
Nanotechnology |
Active Substance |
Indication |
Approved |
Polymeric drugs |
Glatiramer acetate
(Copaxone) |
Multiple sclerosis |
1996 |
Leuprolide Acetate (Eligard) |
Prostate cancer |
2002 |
Liposomes |
Amphotericin B
(Ambisome) |
Fungal Infections |
1990 (EU)
1997 (US) |
Bupivacaine
(Exparel) |
Anaesthetic |
2011 |
Cytarabine
(DepoCyt) |
Meningeal neoplasms |
1999 |
Daunorubicin
(DaunoXome) |
Advanced HIV-associated Kaposi’s sarcoma |
1996 |
Doxorubicin hydrochloride
(Myocet) |
Breast neoplasms |
2000 |
Pegylated Doxorubicin hydrochloride
(Doxil) |
Breast and ovarian neoplasms, multiple myeloma, Kaposi’s sarcoma |
1995 |
Mifamurtide
(Mepct) |
Osteosarcoma |
2009 |
Morphine
(DepoDur) |
Pain relief |
2004 |
Verteporfin
(Visudyne) |
Macular degeneration, myopia (degenerative) |
2000 |
Vincristine
(Marqibo) |
Philadelphia chromosome–negative ALL |
2012 |
Nanocrystals |
Sirolimus
(Rapammune) |
Immunosuppressant for kidney transplant |
2000 |
Aprepitant
(Emend) |
Nausea and vomiting during chemotherapy |
2003 |
Fenofibrate
(Tricor) |
Hyperlipidemia |
2004 |
Paliperidone palmitate
(3-month)
(Invega Sustenna) |
Schizophrenia |
2016 (EU)
2015 (US) |
Paliperidone palmitate
(1-month)
(Invega Sustenna) |
Schizophrenia |
2011 (EU)
2009 (US) |
Nanoparticles |
Ferric carboxymaltose
(Injectafer) |
Iron deficiency |
2007 |
Ferumoxytol
(Feraheme) |
Iron deficiency |
2009 |
HMW iron dextran
(Dexferrum) |
Iron deficiency |
1996 |
Iron gluconate
(Ferrlecit) |
Iron deficiency |
1999 |
Iron sucrose
(Venofer) |
Iron deficiency |
1949 (EU)
1992 (US) |
LMW iron dextran
(INFeD) |
Iron deficiency |
2001 |
Denileukin Deftitox
(Ontak) |
Cutaneous T-cell lymphoma |
1999 |
Paclitaxel
(Abraxane) |
Metastatic breast cancer |
2005 |
Abbreviations: ALL, acute lymphoblastic leukemia; EU, European Union; HMW, high molecular weight; LMW, low molecular weight; US, United States. |
Nanoparticles: Most Common Form of Nanomedicine
There are many different types of nanoparticle (NP)/nanocarrier (NC) systems for medical applications (briefly summarized in TABLE 2),3,21,22 which can be generally classified, based on their composition, into:
- Inorganic: Quantum dots, silica, gold, and iron NPs
- Polymeric: Poly (lactic-co-glycolic acid) (PLGA) NPs, dendrimers, nanospheres, and micelles
- Lipid-based: Liposomes, lipid NPs, and nanoemulsions.3
TABLE 2. Summary of the Properties of Major Nanocarrier Systems Used in Biomedical Applications3,22 |
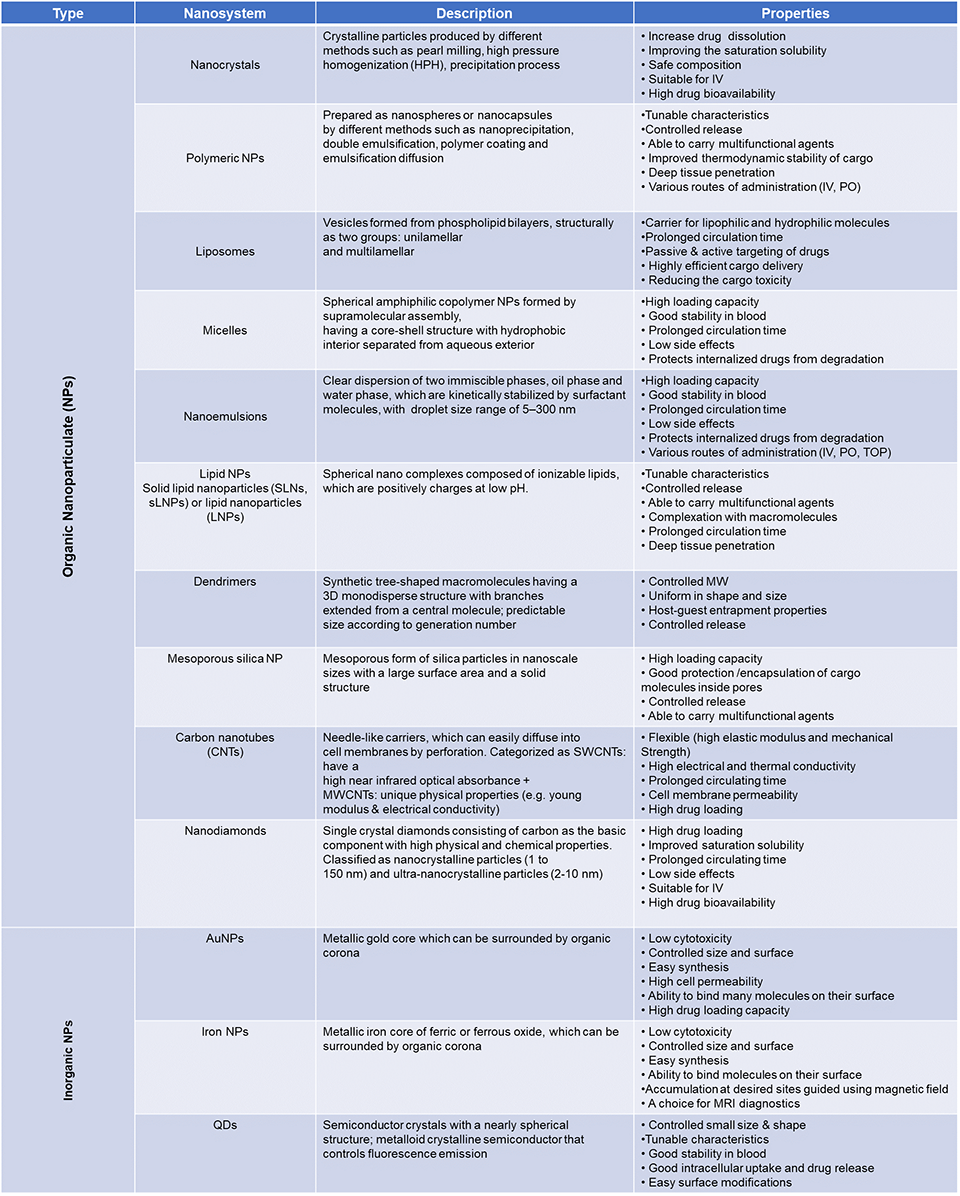 |
Abbreviations: AuNPs, gold nanoparticles; IV, intravenous; MRI, magnetic resonance imaging; MW, molecular weight; MWCNTs, multi-walled carbon nanotubes; PO, oral; QDs, quantum dots; SWCNTs, single-walled carbon nanotubes; TOP, topical. |
Nanocrystals constitute a unique group of nanomedicines since they are entirely composed of water-insoluble drug substances, without any other added nanomaterials or NC. Emend (aprepitant), Rapamune (sirolimus), and Tricor (fenofibrate) are current clinical examples.14,23
In addition, nanoscale bioconjugates have been considered nanomedicines, which include proteins, polypeptides, and aptamers, which have been covalently linked to a varying number of polyethylene glycol (PEG) chains, display a very different pharmacokinetic, and possess a totally altered bioavailability in comparison to their non-PEGylated parent molecules. Examples are Adagen (pegademase bovine), Neulasta (pegfilgrastam), Oncaspar (pegaspargase), and Somavert (pegvisomant).2
Protein-drug conjugates are using common proteins like albumin as NCs as in Abraxane, which is composed of the anticancer drug paclitaxel, linked to albumin NPs.3,13,21
An important group of nanomedicines is based on inorganic/metal NPs, which were well-developed over the past century. Notably, the first iron carbohydrate nanomedicine, Venofer (iron sucrose), was approved back in1949 (in Switzerland/EU), which has now expanded into many product variations and brands for both therapeutic and diagnostic uses.2,3,24
Potential Advantages/Challenges of Nanomedicines Over Conventional Medicines
Owing to their unique nanoscale and structural properties, nanomedicine platforms offer the following physicochemical and biomedical advantages to reformulated conventional drugs3:
- The incorporation of active drugs within a uniform nanometer-particle size range and uniformity, with the specifically controlled geometric shape of NPs, helps enhance the stability of encapsulated material and prevent its direct interaction with biological molecules, which can result in active drug biodegradation or bio-incompatibility (in the form of adverse toxic reactions), prior to reaching its intended target site.
- The loading capacity of nanomedicine platforms, owing to their remarkable porosity, lamellarity, and inherently amazing surface-area-to-size ratio, has been shown to far surpass the simple additive or cosolvency capacity of conventional formulations. Hydrophobicity of small-drug molecules leads to improved permeability through cellular membranes; it has a negative effect on the solubility crossing the aqueous phase in circulating or tissue fluids. The low aqueous solubility of these lipophilic drugs usually causes aggregation and local embolism. Therefore, nanomedicine platforms offer a solution to size-dependent aggregation and crystallization bio-incompatibility issues and adverse effects that can be associated with micronized conventional drug formulations.3
On the other hand, hydrophilic agents—including proteins, peptides, and nucleic acids—are very soluble in body fluids but poorly across the cellular membrane. Over the past 10 years, nucleotide-based therapeutics, such as DNA, short/silencing interfering RNA (siRNA), and microRNA (mRNA), have emerged in preclinical work to regulate certain gene expressions. However, these negatively charged molecules have poor cellular uptake and fast degradation physiologically, limiting their biomedical applications. To solve these problems, various NC systems have been developed, offering improved size-dependent dissolution and permeability for these macromolecules. Cationic liposome and polymeric/lipid NPs are the most common methods to neutralize polyanionic nature of nucleotides for cellular delivery.3,4 Surface modifications of these nano-lipid complexes have been further adjusted to improve their hemocompatibility and biodistribution profiles in vivo.1,24
In 2018, the FDA approved the first lipid nanoparticle (LNP) product, Onpattro (patisiran), consisting of siRNA encapsulated in long-circulating LNP, indicated for polyneuropathy patients with hereditary transthyretin mediated amyloidosis.12-15 Briefly, the ionizable lipid in Onpattro, with its pKA of 6.4 complexes with nucleic acids in acidic media (pH ~4), and yet becomes neutral at physiological pH of 7.4, which significantly reduces interactions with blood components, thus minimizing immunogenicity. Following internalization of Onpattro, its structural and helper lipids become positively charged inside endosomes, thus interacting with lysosomal membrane, and mediating effective delivery the RNA cargo into the target cell cytoplasm. The surface modification of these LNPs with PEG-lipids prevents aggregation, and prolongs their plasma circulation time, similar to the first FDA-approved flagship nanomedicine, Doxil/Caelyx (PEGylated liposomal doxorubicin).12-15
This commercial LNP platform proved the great clinical potential for lipid-based NPs as a particularly promising nanosystem for genetic therapies owing to their higher biocompatibility, structural flexibility, inherent cell-penetrating ability, and low immunogenicity.25,26 These nanoformulations can also be feasibly produced in large scale, which is a major advantage when moving into clinical trials and commercial distribution,25 as has been later seen through the fast-track development of the LNP-based COVID-19 vaccines.
Indeed, there are numerous potential advantages of engineered therapeutic nanomedicines: transforming the unfavorable physicochemical properties of bioactive molecules to desirable pharmacological profiles; improving the delivery of therapeutics across biological barriers and compartments; controlling the release of bioactive agents; enhancing therapeutic efficacy by the selective delivery of therapeutics to biological targets, and combining multimodal imaging and simultaneous diagnosis and therapy in multifunctional nanoplatforms.3,13,26
Still, a detailed understanding of the pharmacologic and toxicologic properties of these nanomaterials, coupled with a balanced and detailed evaluation of their risks and benefits to human health, remains a fundamental challenge before approval of their translation into any clinical indication. Although these materials are likely to provide a high degree of biocompatibility, their complexity in structure and nanoengineering can impact their widespread clinical utilization. Hence, a comprehensive investigation of their short- and long-term interactions with biomacromolecules is fundamental for their clinical translation. Moreover, for safer clinical practice and effective therapeutic monitoring, important safety and efficacy parameters, including systemic biodistribution, plasma half-life (T1/2), hemocompatibility, and minimal off-target effects, along with complete bodily clearance and minimal toxicity to healthy tissues, should be accurately modeled and consistently evaluated, across various nanomedicine systems.21,23
IMPACT OF NANOMEDICINES
Understanding Pharmacodynamics/Pharmacokinetics
The nanoengineered particulate size and structural features of nanomedicine platforms mediate their pharmaceutical actions via one or a combination of the following modes, which involve altering the systemic bioavailability, tissue biodistribution, and pharmacokinetics of the incorporated free drug.
The multiple wall (multilamellar) and porous structures of nanomedicines can be utilized to control the release of the incorporated active drug molecules. For instance, multilamellar liposomes (MLVs) are often utilized as a slow or sustained release drug carrier. MLVs are composed of several bilayer phospholipid membranes, with each membrane surrounding one aqueous compartment suitable for accommodating low molecular weight (LMW) hydrophilic molecules. Following intravenous (IV) administration, the drug loaded MLVs will slowly disintegrate via a variety of mechanisms after reaching their target tissue, gradually releasing the encapsulated drug.
Nanomedicine uptake by the reticuloendothelial system (RES) cells (i.e., macrophages and monocytes) was considered the “natural fate” of plain/unmodified NCs, namely early generation liposomes. The ability to modify the surface of these archetypal NCs, specifically with PEG chains, has allowed them to escape that physiological fate. Surface modification of liposomal doxorubicin (DOX) by chemically coupling PEG2000 chains, creates a steric cloud of PEG surrounding the liposomal DOX carrier, which prevents its interaction and elimination via cells and organs of the RES, thus imparting a stealth character on these parenterally administered NCs. This stealth (PEGylation) technology, which significantly increased the circulation T1/2 of the DOX liposomal nanomedicine, led to the development of Doxil as the first clinically approved nanopharmaceutical in the late 90s.25
The enhanced permeability and retention (EPR) effect is based on defective vascular architecture and impaired lymphatic drainage of solid tumors. In the case of PEGylated NCs, which exhibit sufficiently long plasma circulation T1/2, a small portion will passively accumulate in the interstitial tissue of a solid tumor. Due to the leaky vasculature of tumor tissue, with average cutoff size of the gaps in their endothelial cell lining of about 400 nm, long-circulating nanomedicine will continue to extravasate through and accrue into the tumor tissue over time. This passive tumor-targeting ability of PEG-nanomedicines has led to improved clinical efficacy, as the lower total dose of chemotherapeutic drugs was required to achieve high local concentration at diseased tissue/organ. Consequently, this resulted in reduced nonspecific toxicity and other adverse effects.5,9,12,18
Owing to their high surface area, NPs offer a multi-active substrate for recognizing and interacting with many biomolecular molecules. Direct bioconjugation techniques require NPs with functional groups such as amine, aldehyde, or active hydrogen groups on the surface. For instance, immunoglobins or targeting peptide ligands can directly react with DSPE-PEG-amine or carbonyl phospholipid, to be covalently attached onto the surface of the liposomes.9 Direct functionalization of the nanomedicines’ surface/backbone with a targeting agent/moiety, facilitates active delivery of nanomedicines via coupling high-affinity molecules on their surface to recognize pathological cells directly.26
Active targeting based on surface receptors on target cells has been widely explored since malignant cells upregulate certain tumor-preferred receptors. For example, transferrin receptor (TfR) and folate receptors (FRs) are physiologically expressed on various normal cells but overexpressed in many cancer types in response to their higher metabolic rate.10,11 Both TfR-mediated delivery and folic-acid-conjugated NPs have been reported to have an efficient targeting of the TfR and folate receptors of various tumor types in preclinical tumor models, respectively.24 Moreover, due to the accelerated growth rate of tumor tissues, they often rely on the amplification and mutation of several growth factor proteins, such as the epidermal growth factor receptor (EGFR). EGFR is overexpressed on a variety of primary human cancer cells, and it is involved in tyrosine kinase (TK) signaling pathways that contribute both to tumor initiation and tumor progression. Thus, monoclonal antibodies (mAbs), such as anti-EGFR mAbs (eg, cetuximab/C225), have been under clinical evaluation as immunoliposomes loaded with doxorubicin (ILS-DOX) for efficient targeting of EGFR-positive tumors, such as advanced breast cancer and high-grade gliomas.3,23
Examples of Specific Areas of Impact
Oncology
Even though the past 2 decades have witnessed major advances in cancer diagnosis and treatment, cancer remains the second leading cause of death in the US, and the total number of diagnosed cancer patients is still increasing globally.21 Currently, most of the anticancer chemotherapeutic agents have several clinical drawbacks due to their unfavorable pharmacokinetics and partial tumor-specific effects in the body. Severe treatment-induced toxicities and poor antitumor efficacy (mainly due to development of multidrug resistance in tumors) have become the 2 major hurdles for the success of conventional anticancer chemotherapy. Cancer nanomedicines are quickly progressing and are currently incorporated into clinical practice in order to overcome the clinical limitations often encountered with conventional chemotherapy delivery systems, such as the lack of aqueous solubility, biocompatibility, poor systemic bioavailability, nonspecific biodistribution to healthy tissues, and low therapeutic indices. Furthermore, nanoscale materials have been designed to both overcome the lack of specificity of the original chemotherapeutic agents and to facilitate early detection of the presence of precancerous and malignant lesions.3,27
In order to improve the biodistribution of chemotherapy drugs, a variety of NC systems have been successfully designed and approved for clinical practice, including liposomes and polymers (eg, micelles and protein/polymeric NPs). Overall, the encapsulation of the chemotherapeutic drug within such NC systems (eg, PEG-liposomes), drastically reduces its volume of distribution, thus decreasing its biodistribution within healthy tissue and organs, and consequently reducing its systemic toxicities, especially when administered less frequently than original chemotherapy cycles, all while increasing the active drug accumulation and concentration within the neoplastic tissue. Yet despite the many advantages of all the anticancer nanomedicine, only a few have received FDA approval: 2 polymer-protein conjugates, 5 liposomal formulations, and 1 polymeric NP are available in the market so far, in contrast to the 16 FDA-approved mAbs available.27,28
Starting in 1995, Doxil/Caelyx, the PEGylated liposomal platform of the anthracycline drug doxorubicin, was approved by the FDA as the first nanomedicine for clinical use, indicated for AIDS-associated Kaposi sarcoma tumors.5 This platform involved the encapsulation of doxorubicin hydrochloride in Stealth liposomes (100 nm) composed of N-(carbonyl-methoxypolyethylene glycol 2000)-1,2-distearoyl-sn-glycero3-phosphoethanolamine sodium (MEG2000-DSPE), fully hydrogenated soy phosphatidylcholine, and cholesterol. Doxil’s design allows for passive targeting via EPR, leading to enhanced accumulation of doxorubicin in the sarcoma lesions. Clinically, it has been shown that PEGylated doxorubicin has greatly improved pharmacokinetics, increasing plasma T1/2 to 60 hours, in comparison to only 3 hours for conventional doxorubicin IV infusion, and reduced cardiotoxicity and adverse effects associated with free DOX.18
Furthermore, a recent meta-analysis of 9 randomized controlled trials compared liposomal formulations and conventional doxorubicin, which included 2220 patients (with advanced ovarian, breast, and prostate tumors).29 The primary study outcome was the adverse effects, including congestive heart failure (CHF), hematological toxicity, palmar-plantar erythrodysesthesia (PPE, aka hand-foot syndrome), alopecia, nausea, and vomiting. It was found that liposomal doxorubicin formulations (Lip DOX) have low incidence of CHF (odds ratio [OR] 0.34; 95% confidence interval [CI], 0.24-0.47); alopecia (OR 0.25; 95% CI, 0.10-0.62); neutropenia (OR 0.62; 95% CI, 0.45- 0.85); anemia (OR 0.89; 95% CI, 0.71-1.125); and thrombocytopenia (OR 0.87; 95% CI, 0.61-1.25), though not all reached statistical significance. Such wide analysis confirmed that Lip DOX and PEG Lip DOX demonstrated favorable toxicity profiles with better cardiac safety and less myelosuppression, alopecia, nausea, and vomiting compared with the conventional/free DOX. It was also concluded that the superior therapeutic index of liposomal anthracyclines, without compromising the antitumor efficacy, makes it a favorable choice over conventional anthracyclines in elderly patients, patients with risk factors for cardiac disease, and patients with prior use of anthracyclines.29 Currently, Doxil is approved for recurrent/metastatic breast and ovarian carcinomas, and in association with Velcade (bortezomib), for the treatment of multiple myeloma.3,30
In 1996, DaunoXome (liposomal daunorubicin) was also approved as a first-line therapy for HIV-related Kaposi’s sarcoma by the FDA and the EMA (European Medicines Agency). In a follow-up phase 4 study, DaunoXome demonstrated good tolerability (absence of cardiotoxicity) and effectiveness.3 Furthermore, concomitant administration of highly active antiretroviral treatment (HAART) also proved to be safe.15
Another commercial nanomedicine product, Myocet, consists of doxorubicin citrate encapsulated in non-PEGylated liposome (egg phosphatidylcholine and cholesterol). It is currently approved in combination with cyclophosphamide as a first-line treatment of metastatic breast cancer in adult women.3,16
A notable antitumor nanomedicine is Abraxane, which is comprised of paclitaxel anticancer drug bound to human albumin NP, was approved by the FDA in 2005 for the treatment of metastatic breast cancer in patients who fail to respond to platinum or anthracycline chemotherapy or relapse. In 2012, the FDA expanded the indications for Abraxane to treat non-small cell lung cancer (NSCLC). Since this albumin-bound paclitaxel nanomedicine (average size = 130 nm) formulation eliminates the Cremophor EL surfactant and ethanol cosolvent, which are pharmaceutical excipients in the original Taxol, Abraxane is safely administered in a shorter period of 30 minutes with no need for premedication, compared to Taxol IV infusion.3,30
COVID-19 mRNA Vaccines
While traditional vaccine platforms, such as live attenuated and inactivated virus plus subunit vaccines, have successfully eradicated many infectious diseases for emerging viral pandemic vaccines, the main challenge has become the desperate need for rapid development that cannot be achieved via conventional approaches. Therefore, nucleic acid (DNA- and RNA-based) vaccines present several benefits over conventional protein-based vaccines, including relatively facile synthesis, stability, safety, effective antigen manipulation, and cost-effective scale-up potential.23,31 Nevertheless, nucleic acids carry some inherent disadvantages. DNA has low immunogenicity, requires intranuclear delivery, and might integrate with the human genome. RNA is rapidly degraded in physiological media and rapidly cleared via glomerular filtration.10 Despite that, RNA has been considered a far superior platform for vaccine development against various diseases over the past decade. RNA-based vaccines possess tremendous advantages, including11,23:
- Safety: As a noninfectious or nonintegrating platform, it carries no potential risk of infection or insertional mutagenesis, and no inflammatory adverse effects
- Efficacy: Nucleoside modifications can greatly improve mRNA stability and its translational capacity
- Self-adjuvancy: Amplified RNA serves as self-adjuvant vaccine that can enhance and prolong immune response
- Rapid preparation and versatility: It takes only several days to develop an mRNA vaccine once the gene sequence is identified, and the approach is highly versatile and applicable to almost all protein targets.32
And to overcome the short plasma T1/2 limitation of the RNA-based vaccinees, synthetic RNA molecules are complexed with ionizable lipids, cholesterol, and helper phospholipids as LNPs, rendering the nanoengineered mRNA vaccines more stable and resistant to RNase-mediated degradation.6,7 This optimized LNP composition allow for the disruption of the lipid bilayer to promote the endosomal release of mRNA cargo in the cytosol, where it is translated into antigenic proteins to stimulate effector T cells to generate host antibodies from B cells. Furthermore, lipid-anchored PEGs are deposited on the surface as a barrier to sterically stabilize the LNP-mRNA system and reduce nonspecific binding to proteins. Overall, the LNP platform of mRNA vaccines has been ultimately shown to produce robust adaptive humoral and cellular immune responses.33,34
In response to the global corona virus pandemic, swift development of vaccines for the COVID-19 disease has been a necessity by mid-2020. Taking advantage of versatility and rapid development, 2 COVID-19 mRNA vaccines (mRNA-1273 and BNT162b2) have been approved for market, 1 candidate is in phase 3 clinical trials, and 3 other candidates are currently in phase 1 or 2 clinical assessment.35 Thus far, mRNA-LNPs vaccines has led the global clinical development and were the first fast-track, FDA-approved vaccines against COVID-19. These synthetic mRNA encode for part of the SARS-CoV2 virus spike protein. Specifically, the S protein of SARS-CoV-2 viruses mediates cell attachment, receptor recognition, and fusion for viral penetration and infection.36 On December 11, 2020, the FDA approved the Pfizer-BioNTech COVID-19 Vaccine to be distributed in the US.37 And without much delay, on December 17, 2020, Moderna gained emergency-use authorization from the FDA for their mRNA-1273 vaccine.38 Currently, the BNT162b2 vaccine (Comirnaty), with 2 injections, showed 95% efficacy (95% CI, 90.3-97.6) at preventing COVID-19. Similarly, 2 doses of mRNA-1273 (Spikevax) resulted in efficacy of 94.1% (95% CI, 89.3-96.8) in preventing COVID-19.39 Another COVID-19 mRNA vaccine candidate is now in phase 3 clinical trials, and 3 other candidates are in phase 1 or 2 clinical testings, all utilizing lipid-based NCs.33,40
In the Pipeline
Until now, most of the approved and investigated nanomedicines have been unable to completely fulfill the ultimate magic-bullet promise of specific targeting to diseased tissues or cells.
Passive targeting fits basic clinical applications but still has some limitations. Ubiquitous recognition and off-targeting by passive delivery could cause lower affinity and random binding to the healthy supporting tissue of nearby tumors. In addition, the passive strategy faces the difficulty of reaching circulating tumor cells due to the lack of such permeable mass. There is an intense debate about the real value of the EPR effect clinically. Some studies support the EPR-mediated accumulation of delivery systems within tumors, while others show that this effect depends on the tumor model, suggesting that the EPR effect alone may not provide the entire solution.24,28
Recently, the construction of “smart” or stimulus-responsive delivery vehicles capable of responding to specific internal or external, physical or chemical stimuli has been a growing domain in nanomedicine research.26 Potential targetable stimuli are biochemical changes in pH, the concentration of reducing agent, or ionic strength as notable examples, which have been applied in the design of smart nanomedicines.28,30 This is based on the Warburg effect generally observed in tumor tissue, where a local high metabolic and glycolysis rate results in an acidic environment.7 Designed pH-sensitive nanocarrier could be stable at physiological pH = 7.4, but rapidly disassembled and released payload once it reaches such an acidic tumor microenvironment. A common design of pH-sensitive NP is based on polymers with pKa in the range of 6.5-7.2, such as poly(L-histidine) (PHis). Novel pH-sensitive micelles with PEG-Phis and PEG-PLA polymers that remain stable at physiological pH 7.0-7.4 of plasma and healthy tissues, and disassembled at a pathological pH of 6.6-7.2 at the tumor site, thus making pH-controlled uptake and toxicity tumor-selective delivery of chemotherapy.19,26 Moreover, sophisticated, active-targeting strategies can also be used to carry different drugs into specific targets, which has been widely applied for cancer therapy, and are currently at various clinical phases, where the targeting moieties allow the drug carrier to bind to specific biomarkers that are overexpressed on tumors.26, 26, 30
Based on the carrier type, cargo, and biological target, researchers are currently pursuing either or both strategies in vivo. Smart NCs that sense and respond to acidic pH and higher temperature at tumor sites, or targeted agents that recognize tumor-specific biomarkers while at the same time effectively masking the hydrophobic drug cargos, make them an ideal choice in tumor targeting.36,37 This also helps to increase its blood circulation time and to attain the optimum therapeutic concentration of the drug at the target site, as well as to decrease its accumulation in nontargeted regions through surface modification with biocompatible and biodegradable polymers.41
Stemming from the first-approved nanomedicines (Doxil and Onpattro) and expanding upon the current COVID-19 nanolipid vaccines, LNPs and liposomes showed their best suitability for rapid development of RNA-based vaccines in immunogenic ability, pharmacokinetics, tissue distribution, and targeted delivery to immune cells (namely dendritic cells, T-cells, and macrophages).34,40 Now with multiple candidates at various stages of clinical development, it has become evident that LNPs (with nanostructured or solid cores) have become the most suitable and versatile nano-delivery system for mRNA coding antigens for many anticancer vaccines for prophylaxis, and treatment indications.10,23,31,40
CURRENT STATUS OF NANOMEDICINES
Growth of the Global Nanomedicine Market
Nanomedicines generally have a high added value compared with conventional pharmaceuticals. Although about 100 nanomedicines have been approved by the FDA,15 there has not been a sufficient description of the current market trends in this field. So far, a total of 25 devices and 122 therapeutics are currently in development, accounting for almost 800 ongoing clinical trials.14 It is undeniable that nanomedicines present extraordinary advantages with respect to conventional medicines including offering superior efficacy, bioavailability, improved transport across the biological barriers and disease-targeting, better adsorption, prolonged circulation and blood concentration, reduced toxicity, and immunogenicity.30,42
Presently, a variety of nano-based pharmaceuticals have successfully entered to the market and are utilized every day by many patients (TABLE 1). These products come from various companies all around the world and indicate the present and foreseeable future success of nanomaterials as therapeutic agents.3 With the progressive growth rate of some nanopharmaceuticals and the ever-increasing need for more efficient medicines for treating cancer, immune disorders, and nervous system diseases, as well as mitigating infectious diseases such as AIDS, the worldwide marketplace is expected to show a compound annual growth rate (CAGR) of 22% for the NPs in the life sciences. In a recent market report, it was estimated that “the global nanomedicine market is anticipated to reach USD 350.8 billion by 2025.”30
The global market for nanopharmaceuticals and nanomedicines will continue to develop further over the next decade, mainly thanks to advances in bionanotechnology and nanoengineering, the introduction of clear rules on new nanotechnology-based drugs, more funding from government organizations, more consensus on environmental issues, and formation of partnerships between nanomedicine startups or research and development (R&D) departments and leading medical and pharmaceutical companies.3,42
Review of FDA Approvals
At present, the time scale for a drug to enter the market after its initial discovery/development, may take up to 2 decades. Among other considerations, there should be sufficient skilled scientific and medical personnel willing to spend a decade or 2 of their lives on a single project; the fundamental scientific premise should be novel with appropriate intellectual property protection, and the economic business plan needs to convince the investors about future profits. Market needs should be properly evaluated, and profit/risk ratios should be high enough. The distribution and shelf-life of the therapeutic agents need to be clarified as well. Some key steps along the way include intellectual property, technical issues, and most importantly, the ethics and regulatory affairs of this growing section of health care.42,43
One of the requirements for progress in this field is, nevertheless, a thorough physicochemical characterization of the nanomedicine before in vivo evaluation is carried out. If we examine most of the nanomedicines that have been studied until now, we realize that for the most part, they are nanoformulations of already approved drugs. It is important to remember that nanomaterials possess specific physiochemical properties due to their small size leading not only to the advantages listed above but also to concerns about safety and toxicity. In fact, since nanosystems interact in different ways with biological components, it is vital to test for toxicity using validated methods tailored to the nanomaterials.17,28
Until recently, the FDA had not published any specific guidance document for nanopharmaceuticals (or the category of nanomaterials in general). However, in August 2016, a document addressing general regulations for all nanomaterial products related to cosmetics, food ingredients, and animal feedstuff was issued.17 Since the FDA is not yet entirely convinced that nanopharmaceuticals perform very differently from other small-molecule drugs (except for certain selected characteristics), definite guidance has not yet been issued.42
On the contrary, scientists believe that nanosized materials are not only different from the same bulk material in size and surface area, but particularly in the case of therapeutics, they also differ in biodistribution, toxicity, pharmacokinetics, and excretion profiles.12,17 One of the problems for nanopharmaceuticals is the fact that, since such materials behave differently in various environments (especially in vivo), current testing methods are not able to give clear answers to the above questions. This makes it difficult for companies, which are required to fully test their novel pharmaceuticals before applying for approval. Each pharmaceutical agent should be evaluated for its ultimate application.8 For instance, an injectable drug should be tested in the bloodstream, or a tablet should be evaluated in appropriate solutions to replicate the digestive tract. Because the proprietary process of manufacturing nanomedicines dictates product composition and significantly influences its behavior in vivo, it is nearly impossible to manufacture an exact copy of the reference drug. Since a direct correlation is not predictably found between the physicochemical properties of a nanomedicine and its clinical efficacy and safety, non-clinical and clinical PK, specifically certain tissue uptake, biodistribution, and immune-system interactions must be evaluated. To determine the interchangeability of nanogeneric products (i.e. nanosimilars), therapeutic equivalence of efficacy and safety must be demonstrated in a relevant clinical setting. The appropriate clinical differences of a nanosimilar and the reference nanomedicine product cannot be excluded without the necessary scientific data, including comparative clinical outcome studies. Hence, it is imperative to realize that standard in vitro–in vivo correlations (IVIVC) and bioequivalence methods would not be sufficient when approving similar nanopharmaceutical products, which might require phase 4 trial evaluation and monitoring of nanosimilars.17,44,45
In a case control study of recurrent ovarian cancer, only 4.3% of patients responded to the drug Lipidox (doxorubicin hydrochloride liposome) versus 18% of patients receiving original Doxil (liposomal doxorubicin).46 “However, despite these challenges, many product-specific guidance documents from the FDA do not propose bioequivalence evaluation methods that cover all the challenges outlined in the nanomedicine guidance.”12,44,45
While the guidance of regulatory agencies is important for identifying the information needed for NDAs, the diversity and complexity of the different nanomedicine approaches have been obstructing the realization of a general guidance protocol. In fact, the FDA addresses this point with dedicated industry documents such as “Liposome Drug Products: Chemistry, Manufacturing, and Controls; Human Pharmacokinetics and Bioavailability; and Labeling Documentation (April 2018),” and “Drug Products, Including Biological Products, that Contain Nanomaterials–Guidance for Industry (April 2022).”17
Even today, decades later after the approval of AmBisome and Doxil, nanomaterials and specifically nanopharmaceuticals, is still a young field.5 Over the upcoming period of expansion in nanomedicine, it is imperative that the FDA, EMA, and similar regulatory agencies continue to improve the regulatory frameworks for these materials.17,25
Discussion of the FDA’s Nanotechnology Task Force
In 2006, the Acting Commissioner of Food and Drugs charged the FDA's Nanotechnology Task Force with issuing a report to determine regulatory approaches that would enable the continued development of innovative, safe, and effective FDA-regulated products that use nanoscale materials.4,30,45
With the gradual increase in nanomaterial-containing product submissions to FDA, the Task Force encourages regulatory science research in nanotechnology through internal research grants and provides hands-on nanotechnology training to FDA reviewers and research staff to build scientific expertise and capacity. The Task Force members and subject matter experts at the FDA also are active in international documentary nanotechnology standards development.4,8
While legal standards differ among various product categories that the FDA regulates, the agency will regulate nanotechnology products under existing statutory authorities, in accordance with the specific legal standards applicable to each type of product under its jurisdiction. Therefore, unless specific findings warrant special consideration for a particular
product, the development of nanodrugs follows the typical drug development process.
However, this approach to nanomedicine regulation has been questioned. However, it remains that as a general approach, the FDA will not make broad, general assumptions about the safety of nanotechnology products.30,43
Finally, as the FDA and its Nanotechnology Task Force consider the current safety assessment sufficiently robust and flexible to be appropriate for nanomaterial, the industry remains responsible for ensuring that its products meet all applicable legal requirements, including standards for safety.8 For instance, as was required for accelerated approval of a drug or biological products such as Aduhelm (aducanumab), conducting phase 4 clinical trials could help provide any additional information specifically needed for nanomedicines.45,47 The FDA encourages the industry to consult with the Agency early in the product development process to address any questions related to the safety, and effectiveness of products that contain nanomaterials. This consultation is also encouraged to address any concerns about any impact on the public or the regulatory status of such nanomedicine products, whether as a reference or generic form.30,45
REFERENCES
- Bobo D, Robinson, KL, Islam J, et al. Nanoparticle-based medicines: a review of FDA-approved materials and clinical trials to date. Pharm Res. 2016;33(10):2373-2387. doi:10.1007/s11095-016-1958-5
- Weissig V, Pettinger TK, Murdock N. Nanopharmaceuticals (part 1): products on the market. Int J Nanomedicine. 2014;9:4357-4373. doi:10.2147/IJN.S46900
- Farjadian F, Ghasemi A, Gohari O, et al. Nanopharmaceuticals and nanomedicines currently on the market: challenges and opportunities. Nanomedicine (Lond). 2019;14(1):93-126. doi:10.2217/nnm-2018-0120
- Nanotechnology—Over a Decade of Progress and Innovation: A Report by the U.S. Food and Drug Administration. July 2020. fda.gov/media/140395/download
- Barenholz Y. Doxil—the first FDA-approved nano-drug: lessons learned. J Control Release. 2012;160(2):17-34. doi:10.1016/j.jconrel.2012.03.020
- Kulkarni JA, Witzigmann D, Leung J, et al. On the role of helper lipids in lipid nanoparticle formulations of siRNA. Nanoscale. 2019;11(45):21733-21739. doi:10.1039/c9nr09347h
- Kulkarni JA, Darjuan MM, Mercer JE, et al. On the formation and morphology of lipid nanoparticles containing ionizable cationic lipids and siRNA.ACS Nano. 2018;12(5):4787-4795. doi:10.1021/acsnano.8b01516
- Liu X, et al. Safety considerations of cancer nanomedicine–a key step toward translation.Small. 2020;16(36):e2000673. doi:10.1002/smll.202000673
- ElBayoumi TA, Torchilin VP. Tumor-targeted nanomedicines: enhanced antitumor efficacy in vivo of doxorubicin-loaded, long-circulating liposomes modified with cancer-specific monoclonal antibody. Clin Cancer Res. 2009;15(6):1973-1980. doi:10.1158/1078-0432.CCR-08-2392
- Ickenstein LM, Garidel P. Lipid-based nanoparticle formulations for small molecules and RNA drugs. Expert Opin Drug Deliv. 2019;16(11):1205-1226. doi:10.1080/17425247.2019.1669558
- Huang Q, Zeng J, Yan J. COVID-19 mRNA vaccines. J Genet Genomics. 2021;48(2):107-114. doi:10.1016/j.jgg.2021.02.006
- Smith JA, Mathew L, Burney M, et al. Equivalency challenge: evaluation of Lipodox as the generic equivalent for Doxil in a human ovarian cancer orthotropic mouse model. Gynecol Oncol. 2016;141(2):357-363. doi:10.1016/j.ygyno.2016.02.033
- Pelaz B, Alexiou C, Alvarerz -Puebla RA. et al. Diverse applications of nanomedicine.ACS Nano. 2017;11(3):313-2381. doi:10.1021/acsnano.6b06040
- D'Mello SR, Cruz CN, Chen ML, et al. The evolving landscape of drug products containing nanomaterials in the United States. Nat Nanotechnol. 2017;12(6):523-529. doi:10.1038/nnano.2017.67
- Rosenthal E, Poizot-Martin I, Saint-Marc T, et al. Phase IV study of liposomal daunorubicin (DaunoXome) in AIDS-related Kaposi sarcoma. Am J Clin Oncol. 2002;25(1):57-59. doi:10.1097/00000421-200202000-00012
- De Araújo Lopes SC, dos Santos Giuberti C, Ribeiro Rocha TG, et al. Liposomes as carriers of anticancer drugs. In: Rangel L, ed. Cancer Treatment: Conventional and Innovative Approaches. InTech Open; 2013:85-124.
- Muhlebach S. Regulatory challenges of nanomedicines and their follow-on versions: a generic or similar approach? Adv Drug Deliv Rev. 2018;131:122-131. doi:10.1016/j.addr.2018.06.024
- Fukuda A, Tahara K, Hane Y, et al. Comparison of the adverse event profiles of conventional and liposomal formulations of doxorubicin using the FDA adverse event reporting system. PLoS One. 2017;12(9):e0185654. doi:10.1371/journal.pone.0185654
- Sato Y, Hatakeyama H, Sakurai Y, et al. A pH-sensitive cationic lipid facilitates the delivery of liposomal siRNA and gene silencing activity in vitro and in vivo. J Control Release. 2012;163(3):267-276. doi:10.1016/j.jconrel.2012.09.009
- Sinko PJ. Drug delivery systems and drug product design. In: Sinko PJ, ed. Martin's Physical Pharmacy and Pharmaceutical Sciences. Lippincott Williams & Wilkins; 2016:642-692.
- Chiang CL, Cheng MH, Lin CH. From nanoparticles to cancer nanomedicine: old problems with new solutions. Nanomaterials (Basel). 2021;11(7):1727. doi:10.3390/nano11071727
- Al-Zoubi MS, Al-Zoubi RM. Nanomedicine tactics in cancer treatment: challenge and hope. Crit Rev Oncol Hematol. 2022;174: doi:10.1016/j.critrevonc.2022.103677
- Thi TTH, Suys EJA, Lee JS, et al. Lipid-based nanoparticles in the clinic and clinical trials: from cancer nanomedicine to COVID-19 vaccines. Vaccines (Basel). 2021;9(4):359. doi:10.3390/vaccines9040359
- Weissig V, Guzman-Villanueva D. Nanopharmaceuticals (part 2): products in the pipeline. Int J Nanomedicine. 2015;10:1245-1257. doi:10.2147/IJN.S65526
- Liposomal Doxorubicin Market Size, Share, Industry Report, 2013-2024. Market Analysis Report; 2016. Accessed January 4, 2021. https://grandviewresearch.com/industry-analysis/liposomal-doxorubicin-market
- Mitchell MJ, Billingsley MM, Haley RM, et al. Engineering precision nanoparticles for drug delivery. Nat Rev Drug Discov. 2020;20(2):101-124. doi:10.1038/s41573-020-0090-8
- Kopeckova K, Eckschlager T, Sirc J, et al. Nanodrugs used in cancer therapy. Biomed Pap Med Fac Univ Palacky Olomouc Czech Repub. 2019;163(2):122-131. doi:10.5507/bp.2019.010
- Bisso S, Leroux JC. Nanopharmaceuticals: a focus on their clinical translatability. Int J Pharm. 2020;578: doi:10.1016/j.ijpharm.2020.119098
- Rafiyath SM, Rasul M, Lee B, et al. Comparison of safety and toxicity of liposomal doxorubicin vs. conventional anthracyclines: a meta-analysis. Exp Hematol Oncol. 2012;1(1): doi:10.1186/2162-3619-1-10
- Ventola CL. Progress in nanomedicine: approved and investigational nanodrugs. P T. 2017;42(12):742-755. PMC5720487
- Samaridou E, Heyes J, Lutwyche P. Lipid nanoparticles for nucleic acid delivery: current perspectives. Adv Drug Deliv Rev. 2020;154-155:37-63. doi:10.1016/j.addr.2020.06.002
- Mashel TV, Tarakanchikova YV, Muslimov AR, et al. Overcoming the delivery problem for therapeutic genome editing: current status and perspective of non-viral methods. Biomaterials. 2020;258:120282. doi:10.1016/j.biomaterials.2020.120282
- Jadaan SA, Khan AW. Recent update of COVID-19 vaccines. Adv Pharm Bull. 2022;12(2):219-236. doi:10.34172/apb.2022.045
- Weiss C, Carriere M, Fusco L, et al. Toward nanotechnology-enabled approaches against the COVID-19 pandemic. ACS Nano. 2020;14(6):6383-6406. doi:10.1021/acsnano.0c03697
- Brown S, Brown T, Cederna PS, Rohrich RJ. The race for a COVID-19 vaccine: current trials, novel technologies, and future directions. Plast Reconstr Surg Glob Open. 2020;8(10):e3206. doi:10.1097/GOX.0000000000003206
- Shih HI, Wu CJ, Tu YF, Chi CY. Fighting COVID-19: a quick review of diagnoses, therapies, and vaccines. Biomed J. 2020;43(4):341-354. doi:10.1016/j.bj.2020.05.021
- Tanne JH. Covid-19: Pfizer-BioNTech vaccine is rolled out in US. 2020;371:m4836. doi:10.1136/bmj.m4836
- Ledford H. Moderna COVID vaccine becomes second to get US authorization. Nature. 2020 Dec 18 [online ahead of print]. doi:10.1038/d41586-020-03593-7
- Thompson MG, Burgess JL, Naleway AL et al. Interim estimates of vaccine effectiveness of BNT162b2 and mRNA-1273 COVID-19 vaccines in preventing sARS-CoV-2 infection among health care personnel, first responders, and other essential and frontline workers—eight U.S. locations, December 2020-March 2021. MMWR Morb Mortal Wkly Rep. 2021;70(13):495-500. doi:10.15585/mmwr.mm7013e3
- van Riel D, de Wit E. Next-generation vaccine platforms for COVID-19. Nat Mater. 2020;19(8):810-812. doi:10.1038/s41563-020-0746-0
- Cai Y, Chan X, Si J, et al. All-in-one nanomedicine: multifunctional single-component nanoparticles for cancer theranostics. Small. 2021;17(52):e2103072. doi:10.1002/smll.202103072
- Shi J, Kantoff PW, Wooster R, Farokhzad OC. Cancer nanomedicine: progress, challenges and opportunities. Nat Rev Cancer. 2017;17(1):20-37. doi:10.1038/nrc.2016.108
- Tyner KM, Zheng N, Choi S, et al. How has CDER prepared for the nano revolution? A review of risk assessment, regulatory research, and guidance activities. AAPS J. 2017;19(4):1071-1083. doi:10.1208/s12248-017-0084-6
- Weissig V, Elbayoumi T, Fluhmann B, Barton A. The growing field of nanomedicine and its relevance to pharmacy curricula. Am J Pharm Educ. 2021;85(8):8331. doi:10.5688/ajpe8331
- Astier A, Barton Pai A, Bissig M, et al. How to select a nanosimilar. Ann. N.Y. Acad. Sci. 2017; 1407: 50-62. doi.org/10.1111/nyas.13382
- Smith JA, Costales AB, Jaffari M, et al. Is it equivalent? Evaluation of the clinical activity of single agent Lipodox compared to single agent Doxil in ovarian cancer treatment. J Oncol Pharm Pract. 2016;22(4):599-604. doi:10.1177/1078155215594415
- A Phase 3b/4 Multicenter, Randomized, Double-Blind, Placebo-Controlled, Parallel-Group Study to Verify the Clinical Benefit of Aducanumab (BIIB037) in Participants with Alzheimer's Disease. ClinicalTrials.gov identifier: NCT05310071. Updated September 8, 2022. Accessed September 14, 2022. https://clinicaltrials.gov/ct2/show/record/NCT05310071
Back to Top