Vascular Perfusion and Subcutaneous Space
Unlike humans, carnivores such as dogs and cats typically have large, vascular subcutaneous spaces under loose skin. This anatomical feature helps to guard against serious injury to underlying muscle and hasten healing if the animal is bitten by live prey that it captures as food. The large subcutaneous spaces also can accommodate large fluid volumes, enabling owners of dogs and cats to administer drugs and fluids easily by the subcutaneous route without ever having to develop venipuncture skills. This is particularly useful for dogs and cats that develop renal insufficiency; owners can rehydrate their pets at home with several hundred milliliters of subcutaneous fluid (as instructed by the attending veterinarian) instead of taking pets to the veterinary clinic for intravenous fluids every few days. Many cats that resist administration of unpalatable oral drugs (eg, clindamycin) do not object to subcutaneous injections. Indeed, the subcutaneous route can be an ideal alternative whenever oral administration is not feasible—for example, in animals with mandibular fractures, cervical spine disorders, or oral neoplasia.
Herbivores such as horses and cattle do not have a large subcutaneous space, but they do have large superficial veins that can be accessed easily by trained caregivers for administration of intravenous drug and fluid therapy.
Clinical Pearl
Prescriptions for drugs and fluids that caregivers will administer to animal patients typically call for volumes and routes of administration that pharmacists and pharmacy technicians rarely, if ever, encounter in prescriptions for human outpatients.
|
Gastrointestinal Tract
There are significant anatomical differences between humans and non-human species in the structure and function of the GI tract and the corresponding influence of diet on GI characteristics.11 In addition, gastric pH and average GI transit time (from mouth to anus) vary widely across species (Table 2).
Table 2. Comparative Gastrointestinal Characteristics in Selected Species |
Species |
Typical Gastric pH |
Typical Gastrointestinal
Transit Time (h) |
Fed |
Fasted |
Human |
4-5 |
1.2 |
30–40 |
Dog |
1.1 |
2.0 |
7–9 |
Cat |
2.5 |
2.1 |
26–35 |
Horse |
5.3 |
5.2 |
72–75 |
Cattle |
3 |
3 |
72–78 |
Sources: References 12-17. |
Like humans, cats and dogs are monogastric species (ie, they have a simple, single-chambered stomach). However, GI transit time is somewhat shorter in cats and considerably shorter in dogs than it is in humans, and the stomach contents are about 10 times more acidic due to increased gastric secretion of hydrochloric acid.18 Human gastric pH typically is in the range of 4 to 5—a pH at which bacteria such as E. coli, Campylobacter, and Salmonella species can survive. Humans must cook or acidify their food to decrease risk of food poisoning. Gastric pH in carnivores can drop as low as 1 when food is in the stomach, to facilitate digestion of meat and kill pathogenic bacteria that might be ingested through raw food.19 Although it is possible for carnivores to suffer infection from contaminated ingesta (food or medications), such infections are far less likely than they are in humans.
Differences in pH and average GI transit time have important implications for drug therapy. The low gastric pH in carnivores may increase the bioavailability of drugs that are weak acids. Enteric-coated dosage forms designed to release their contents in the distal GI tract of humans most likely will release in the duodenum of cats, where the pH (5.7) approximates that of the distal human colon.20 Consequently, enteric-coated dosage forms designed for humans are not likely to achieve the desired effect in cats. Also, because GI transit time is much shorter in dogs than it is in humans, delayed-release wax matrix tablets designed to release drugs during the long journey through the human GI tract likely will be excreted in the feces of a dog before any drug is released.
Clinical Pearl
Sustained-release or extended-release dosage forms designed for the human GI tract are unlikely to have the same release profile in non-human patients. Drug contents may be released prematurely or not at all, depending on pH and GI tract length.
|
Horses and rabbits also are monogastric species. However, compared with cats and dogs, they have a longer GI tract and a more developed hindgut (cecum) in which large populations of microflora break down the herbivorous diet of grasses and other fibrous plants into nutritionally valuable carbohydrates.21 Herbivore saliva is alkaline due to the presence of enzymes that facilitate digestion of carbohydrates, so drugs with higher pKa values may be bioavailable when administered via buccal routes. GI transit times in hindgut fermenters are considerably longer than they are in humans, dogs, or cats, and some drugs are vulnerable to degradation during long transit times. Certain drugs may be inactivated by cecal microflora. Alternatively, some antibiotics (eg, macrolides) should be avoided entirely in hindgut fermenters, because they decimate beneficial microflora and can lead to endotoxemia from overgrowth of gram-negative bacteria.22
Ruminant animals such as cattle and sheep have complex stomachs with four separate chambers to facilitate digestion: rumen, reticulum, omasum, and abomasum. The first three compartments (rumen, reticulum, and omasum) are colonized by microflora that digest complex carbohydrates and produce volatile fatty acids. The slightly acidic pH (5.5-6.5) in the rumen and reticulum favors absorption of nonionized forms of weakly acidic drugs; however, the bioavailability of many drugs is reduced substantially by passage through the rumen due to degradation or long residence times (eg, prednisolone).22 The last compartment, the abomasum, is most similar to the stomach in monogastric species. Abomasal pH typically is around 3, which is comparable to gastric pH in monogastrics.11 Absorption of weak acids is favored in the abomasum.
GESTATION
Approximate gestational periods vary considerably by species (Table 3). Although animal pregnancies are not typically divided into trimesters, the known gestational periods can be divided by 3 to draw comparisons with human pregnancy trimesters.
Table 3. Comparative Average Gestational Period and Trimester Length in Selected Species |
Species |
Gestational Period |
Estimated Trimester
Length |
Human |
10 months |
93 days |
Dog |
63 days |
21 days |
Cat |
63 days |
21 days |
Horse |
11 months |
103 days |
Cattle |
10 months |
93 days |
Rabbit |
31 days |
10 days |
Before any drug is used in a pregnant woman, its pregnancy category and safety data are considered carefully, based on the trimester in which the drug will be used. If safety information regarding the use of a particular drug in a pregnant animal is not available—but safety data exist for its use in a pregnant woman—the human safety data should be extrapolated to the pregnant animal. For example, folate antagonists cause congenital malformations in human fetuses when used during the first trimester of pregnancy. It stands to reason that folate antagonists should not be administered to animals during the first trimester of pregnancy.
VITAL SIGNS
Normal vital signs vary significantly from species to species. It is important for pharmacists and pharmacy technicians to be familiar with normal body temperature, heart rate, and respiratory rate in different types of animals, so they can recognize clinically important variations and counsel pet owners about appropriate monitoring parameters.
Temperature
Fever can be a sign of infection or an adverse drug effect. However, a body temperature that would be considered a fever in humans may be considered normal temperature, or even a low normal temperature, in a cat or rabbit. For example, a temperature of 102°F is considered normal in a cat, and unless cat owners are given a threshold for what temperature constitutes a fever, they may be concerned with a temperature of 102°F. Figure 1 depicts comparative values for what most veterinarians consider to be clinically significant fever by species.
Figure 1. Comparative Fever Thresholds in Selected Species
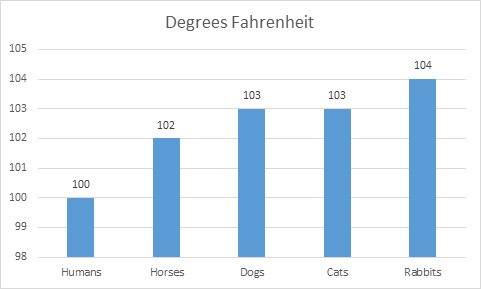 |
Heart Rate and Respiratory Rate
In some instances, pet owners may need to monitor their pets for abnormalities in heart rate (ie, bradycardia or tachycardia) or breathing (ie, dyspnea or tachypnea). Those thresholds typically are very different in animals than they are in humans. For example, a resting heart rate of 60 beats per minute (bpm) would not be cause for concern in a healthy human. In contrast, the normal resting heart rate for a horse typically is 28 to 40 bpm. A horse with a resting heart rate of 60 bpm should be evaluated by a veterinarian immediately, especially if the animal exhibits other signs of discomfort or distress. Owners of cats with cardiomyopathies are counseled to monitor their pet’s pulse to ensure that it ideally stays below 150 bpm.23
Comparative heart rate thresholds for various species are shown in Figure 2. Normal respiratory rates are listed in Table 4.
Table 4. Normal Respiratory Rates in Selected Species |
Species |
Normal Respiratory Rate (breaths/min) |
Human |
12-18 |
Dog |
18-34 |
Cat |
16-40 |
Horse |
10-14 |
Cow |
26-50 |
Rabbit |
30-60 |
Source: Reference 24. |
Figure 2. Comparative Heart Rate Thresholds in Selected Species
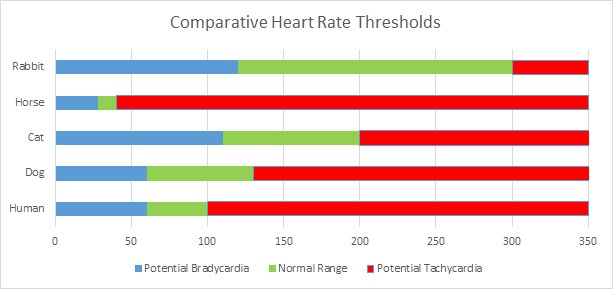 |
DRUG DISPOSITION
Important considerations for drug disposition in non-human species include body composition, protein binding, blood volume, metabolism, urinary pH, glomerular filtration rate, and variations in first-pass effect.
Distribution
Distribution of drugs in non-human species is affected significantly by body composition. Compared with humans, some animals (eg, marine mammals) normally have a greater body adipose tissue percentage, and some (eg, Greyhound dogs) normally have a lower one. Lipophilic drugs that distribute to adipose tissue (eg, thiobarbiturates) will remain in the bloodstream of lean animals, causing dangerously high levels of drug and abnormally long recovery times. Individual animals may undergo dramatic fluctuations in adipose tissue in response to hormonal, seasonal, or dietary variations (eg, egg-laying birds, hibernating mammals, starved animals); all of these conditions can affect lipophilic drug distribution.
Unbound drugs in plasma generally are free to enter tissues and exert an effect, while protein bound drugs generally have a longer duration of effect. Differences in protein binding among species can account for substantial differences in potency and duration of action of certain drugs. For example, the long-acting cephalosporin antibiotic cefovecin is highly protein bound (98.5%–99.8%);25 it is approved for administration once every 14 days to dogs and once every 7 days to cats. In non-human primates, the half-life of cefovecin is only a few hours, presumably because of a much lower degree of protein binding.26
Blood volume per unit of body weight also varies widely across species and contributes to differences in dosage and frequency of administration for non-human species. If all other factors are equal, peak blood concentrations following intravenous administration of a drug (on a mg/kg body weight basis) will be dependent on the blood volume per kilogram of the animal. Table 5 lists approximate values for blood volume per weight by species and the likely relationship to maximum blood concentrations achieved after intravenous administration to humans.
Table 5. Blood Volume in Selected Species |
Species |
Blood Volume (mL/kg) |
Relationship to Human
Cmaxa |
Human |
70 |
--- |
Dog |
70–110b |
Same or ↓ |
Cat |
55 |
↑ |
Horse |
75 |
↓ |
Cow |
60 |
↑ |
Rabbit |
60 |
↑ |
a All other considerations equal.
b Breed-dependent.
Source: Reference 27. |
Metabolism
Variation in drug metabolism is the most important aspect of comparative drug disposition for pharmacists, pharmacy technicians, and veterinary technicians filling prescriptions for non-human patients or consulting with veterinarians.
Phase I Metabolism. Hepatic microsomal enzymes vary significantly across species and may be affected by diet, gender, or age. It is important to note that cytochrome P-450 (CYP) nomenclature is based on species-specific amino acid sequences. It is not possible for non-human species to share a named microsomal enzyme with humans. For example, although CYP3A4 (human) may be functionally similar to CYP3A12 (canine), they are not the same enzyme because of differences between the human and canine amino acid sequences.
Extrapolations from human cytochrome P-450 mediated drug metabolism may or may not accurately predict drug elimination or drug interactions in non-human species. Although rabbits once served as a primary laboratory model for preclinical human drug studies, rabbits do not have a counterpart to the human CYP2D6 microsomal enzyme that is involved in the metabolism of about one-third of drugs used in humans.28 Conversely, dogs share many cytochrome P450 enzyme subfamilies with humans (eg, 3A), and these similarities can be used to predict both useful and harmful drug interactions. For example, cyclosporine A is cleared by the CYP3A subfamily of microsomal enzymes in both dogs and humans. Cyclosporine A is used widely in dogs for a variety of indications, but it is expensive and causes many dose-related adverse effects. Veterinarians often will take advantage of the well-documented drug interaction between ketoconazole and cyclosporine to boost blood levels of cyclosporine in dogs, allowing administration of smaller systemic doses.29
Phase II Metabolism. Differences in phase II metabolism (conjugation) across species are the most well-known and best documented. There are seven major metabolic conjugation reactions in mammals: glucuronidation, sulfation, methylation, acetylation, glutathione conjugation, amino acid conjugation, and fatty acid conjugation.22 Four of these reactions may be deficient or absent in non-human species:
- Glucuronidation
- Sulfation
- Methylation
- Acetylation
It is commonly stated that cats lack the ability to conjugate with glucuronide. This is not completely true; cats do have the ability to conjugate with glucuronide, but lack some of the major glucuronyl transferase enzymes. Domestic cats are unable to conjugate phenol, morphine, acetaminophen, carprofen, some benzodiazepines, and propofol, but they are able to conjugate endogenous substances such as bilirubin, some steroids, and thyroxine.30 Pharmacists, pharmacy technicians, and veterinary technicians should be aware of drug classes that are metabolized through conjugation with glucuronide (eg, opioids, benzodiazepines, steroids, acetaminophen, salicylates, nonsteroidal anti-inflammatory drugs) and recognize that if these drugs are used at all in cats, the dose may be substantially smaller than the human dose, or the drug may be administered less frequently.
Differences in demethylation and hydroxylation also have been described in cats and are responsible for serious hepatotoxicity that may arise following administration of chloramphenicol or oral diazepam.31 Oral diazepam therapy can cause idiosyncratic hepatic failure in some cats; if the benefit of oral diazepam therapy outweighs risk in a feline patient, serum chemistries should be performed 5 days after therapy is initiated to monitor for elevated transaminase.32
Clinical Pearl
Chronic administration of oral diazepam in cats can cause fulminant hepatic failure. Serum transaminase levels should be checked 5 days after initiation of oral diazepam therapy, and therapy should be discontinued if levels are elevated.
|
Many drugs that are conjugated with glucuronide also are cleared through sulfation. Conjugating agents for glucuronidation are abundant in most species, but the endogenous moieties to conjugate with sulfate are in low supply. For example, pigs have a very low capacity for synthesizing sulfate substrates, so the primary conjugation reaction in pigs is glucuronidation.
Acetylation involves transfer of an acetyl group from acetyl coenzyme A to the amino group of the metabolized drug. This can transform a drug into an active metabolite or (more commonly) render it pharmacologically inactive for renal elimination. Because canine species lack specific transacetylase enzymes, acetylation is completely absent in dogs. The inability to acetylate has several major consequences:
- Failure to produce active metabolites necessitates more frequent administration of affected drugs (eg, N-acetylprocainamide)
- More rapid excretion of the parent drug necessitates more frequent administration of affected drugs
- Inability to detoxify certain drugs (eg, isoniazid) increases susceptibility to drug toxicity
On the positive side, the inability to acetylate sulfanilamide to the less soluble and more toxic acetylsulfanilamide tends to protect dogs from the renal toxicity of sulfanilamide.33
Methylation involves the transfer of a methyl group from methionine to the metabolized drug. It is a relatively minor pathway for the biotransformation of drugs. One important deficiency in methylation is seen in cats. Because cats lack thiopurine methyltransferase, azathioprine and 6-mercaptopurine can be quite toxic.30
Table 6 describes species deficiencies in conjugation capability and the targeted functional groups and important drugs for each conjugation reaction.
Table 6. Metabolic Limitations and Affected Drugs in Selected Species |
Species |
Deficient Metabolic
Reaction |
Targeted Functional
Groups |
Drugs Affected |
Cat |
Glucuronidation
Methylation
(deficient in thiopurine
methyltransferase) |
-OH, -COOH,
NH2, =NH, -SH
moieties attached to either phenolic (ring)
or alcoholic (straight-chain)
--SH (purines) |
Acetaminophen
Benzodiazepines
Benzyl alcohol
Benzoic acid derivatives
Carprofen
Chloramphenicol
Codeine
Morphine
Lamotrigene
Phenazopyridine
Phenols
Propofol
Salicylates
Zidovudine
Azathioprine
6-mercaptopurine |
Pig |
Sulfation |
Aromatic—OH,
Aromatic—NH2 |
Acetaminophen
Diflunisal |
Dog |
Acetylation (absent) |
Aromatic—NH2 |
Dapsone
Hydralazine
Isoniazid
Procainamide
Sulfonamides |
Source: Reference 34. |
Elimination
Metabolism of drugs usually produces a more water-soluble metabolite that can be eliminated easily by urinary excretion. The pH of human urine generally is in the range of 5 to 7, favoring the reabsorption of weak acids but promoting final elimination of many drugs. Drugs that typically are excreted or reabsorbed through renal tubules in humans may have a very different elimination profile in other species. Table 7 lists the typical urinary pH for selected mammalian species and the general impact on drug disposition.
Table 7. Urinary pH in Selected Species |
Species |
Typical Urinary
pH |
Residence Time of Drugs Eliminated by
Renal Excretiona |
Weak Acids |
Weak Bases |
Human |
5–7 |
--- |
--- |
Dog |
5.5–7 |
↓ |
↑ |
Cat |
5.5–7 |
↓ |
↑ |
Horse |
7–9 |
↓ |
↑ |
Cattle |
8–9 |
↓ |
↑ |
a Relative to humans.
Source: Reference 35. |
The glomerular filtration rate (GFR) is another critical aspect of drug disposition and monitoring. For drugs and active metabolites that are eliminated via renal excretion, the GFR can dramatically affect mean residence time and half-life—and, subsequently, the dose and frequency of administration of drugs. Logically, the higher the GFR of a given species is compared with human GFR, the less time the drug will persist in that animal’s system relative to humans.
As an example of this concept, canine GFR is approximately 3 times greater than human GFR. The daily dosage of levothyroxine in dogs (0.02 mg/kg/d) is approximately 10 to 15 times higher than the human dosage. This is due largely to decreased oral bioavailability in dogs, but also to faster renal elimination in dogs vs humans.
Table 8 shows the average GFR and predicted effect on the half-life of renally eliminated drugs for selected species.
Table 8. Average Glomerular Filtration Rate in Selected Species |
Species |
Average GFR
(mL/kg/min) |
Predicted Effect on
Half-Life of Renally
Eliminated Drugsa |
Human |
1.3b |
--- |
Dog |
3.96 |
↓↓↓ |
Cat |
2.94 |
↓↓ |
Horse |
1.65 |
↓ |
Cattle |
2.25 |
↓↓ |
a Relative to humans.
b 90 mL/min/1.73m2.
Source: Reference 36. |
Humans and other mammals have a hepatic portal system, in which drugs and nutrients are directed to the liver via blood vessels draining the gut. Pharmacists and pharmacy technicians are aware of the significant effect of hepatic first pass on bioavailability, toxicity, and dosage regimens for drugs in humans; the hepatic first-pass effect is similar in non-human mammals. In addition, birds, amphibians, reptiles, and some fish also have a renal-portal system that returns blood directly to the kidneys from the hindlimbs and/or caudal body, theoretically resulting in a first-pass effect in the kidneys.34 In birds and reptiles, drugs that may be nephrotoxic (eg, aminoglycosides) or renally eliminated (eg, cephalosporins) have thus typically been injected in the anterior portion of the body (eg, the pectoral muscles for birds or the forelimbs of turtles) to avoid nephrotoxicity or rapid elimination before therapeutic effect is achieved. Some drugs, including opioids, aminoglycosides, and beta-lactam antimicrobials undergo a significant hepatic first-pass effect or a significant renal portal system effect after being injected into the hindlimb of reptiles.37,38 Nevertheless, if a veterinarian prescribes injection in a specific region on a given animal patient, pharmacists, pharmacy technicians, and veterinary technicians should emphasize the importance of administering the drug in that site.
SPECIES PHARMACOGENETICS
Long before veterinary pharmacogenetics was an active field of research or a viable clinical approach, veterinarians often followed the adage “white feet, don’t treat” when dosing certain drugs, including chemotherapy agents, antidiarrheals, and heartworm preventatives. Herding canine breeds (many of which have white feet) were known to suffer severe, often fatal, central nervous system toxicity from typical doses of drugs that were not observed to be toxic in other breeds. It is now well established that this increased susceptibility to drug toxicity in certain breeds of dogs is caused by a genetic polymorphism in the ATP binding cassette (ABC) transporter protein, also known as the p-glycoprotein (P-gp) transporter protein, resulting in failure of the P-gp pump at the blood–brain or other blood–compartment barriers.39 A four-base pair deletion mutation in the ABCB1 gene occurs at a very high incidence in many herding breed dogs, including Collies, Australian Shepherds, and Shetland Sheepdogs. The problematic chemotherapy agents (doxorubicin and vinca alkaloids), antidiarrheal (loperamide), and macrocyclic lactone heartworm preventatives (avermectins and milbemycin) all are substrates for P-gp.
The approximate frequency of genetic polymorphism of the ABCB1 gene in high-risk dog breeds is shown in Table 9. For mixed-breed dogs, genetic testing is easily accessible at a nominal fee—a simple cheek swab can determine a dog’s risk for multidrug sensitivity due to genetic polymorphisms. Pharmacists, pharmacy technicians, and veterinary technicians should know which drugs are likely to cause adverse events in susceptible breeds (Table 10) and perform drug use evaluation accordingly. Health care professionals providing pharmaceutical products for dogs should also encourage dog owners to learn more about this important issue by referring them to the Canine Multidrug Sensitivity Testing website (http://vcpl.vetmed.wsu.edu/).
Table 9. Dog Breeds Affected by the ABCB1 P-Glycoprotein Mutation |
Breed |
Approximate Frequency of Mutation (%) |
Australian Shepherd (standard and mini) |
50 |
Border Collie |
< 5 |
Collie |
70 |
English Shepherd |
15 |
German Shepherd |
10 |
Herding Breed Crosses |
10 |
Long-Haired Whippet |
65 |
McNab |
30 |
Mixed breeds |
5 |
Old English Sheepdog |
5 |
Shetland Sheepdog |
15 |
Silken Windhound |
30 |
Source: Reference 40. |
Clinical Pearl
Loperamide causes serious CNS depression in dogs with the ABCB1 mutation. Pharmacists, pharmacy technicians, and veterinary technicians should strongly discourage the use of nonprescription loperamide in dogs for any reason outside of a veterinarian’s order.
|
Genetic deficiencies in protein transporters also may occur at the species level. For example, the unique susceptibility of cats to dose-related retinal toxicity from the fluoroquinolone antibiotic enrofloxacin recently was attributed to failure of the ABCG2 blood–retinal barrier protein transporter. Failure of the ABCG2 P-gp pump in cats results in accumulation of photoreactive fluoroquinolones in the retina;41 exposure of the retina to natural light (through the pupil) generates reactive oxygen species that cause retinal degeneration and blindness. Pharmacists, pharmacy technicians, and veterinary technicians should consider the possibility that pharmacological inhibition of ABCG2—in cats in particular, but also in other species (through genetic polymorphism or drug interactions)—could result in retinal damage if photoreactive drugs are administered concomitantly. Table 10 lists drugs that have the potential to cause adverse effects in animal patients with genetic polymorphisms in P-gp protein transporter pumps.
Table 10. Drugs Known to Cause Adverse Effects in Animals with P-Glycoprotein Mutations
|
Cats
Dogs
- Acepromazine
- Butorphanol
- Chemotherapy agents
- Doxorubicin
- Paclitaxel
- Vinblastine
- Vincristine
- Loperamide
- Macrocyclic lactones
- Ivermectin
- Milbemycin
- Moxidectin (used in doses higher than labeled for heartworm prevention)
- Selamectin
|
Clinical Pearl
Cats lack a competent ABCG2 blood–aqueous barrier. As a result, enrofloxacin is able to enter the retina, where it is photoactivated to toxic oxygen species that cause blindness. Many safer fluoroquinolone antibiotics are approved for use in cats; use of enrofloxacin is strongly discouraged.
|
REFERENCES
- Arnish CE, Davidson GS, Royal K. Veterinary pharmacy education: prevalence and perceptions. Poster presented at: Society of Veterinary Hospital Pharmacists 34th Annual Meeting; June 14-17, 2015; Portland, ME.
- Cima G. Substitution errors: Surveys describe harm from differences between prescriptions and drugs dispensed. J Am Vet Med Assoc. 2014;245(5):462-482.
- Riviere JE, Martin-Jimenez T, Sundlof SF, et al. Interspecies allometric analysis of the comparative pharmacokinetics of 44 drugs across veterinary and laboratory animal species. J Vet Pharmacol Ther.1997;20:453-463.
- American Veterinary Medical Association. Pet ownership down slightly, but spending up. JAVMA News. May 1, 2015. https://www.avma.org/News/JAVMANews/Pages/150501l.aspx. Accessed July 27, 2016.
- Graham JP, Lipman AH, Newell SM, et al. Esophageal transit of capsules in clinically normal cats. Am J Vet Res. 2000;61(6):655-657.
- Westfall DS, Twedt DC, Steyn PF, et al. Evaluation of esophageal transit of tablets and capsules in 30 cats. J Vet Intern Med. 2001;15(5):467-470.
- Nelson R, Couto C, eds. Small Animal Internal Medicine. 5th ed. St. Louis, MO: Elsevier Mosby; 2003.
- Hendricks JC. Brachycephalic airway syndrome. Vet Clin North Am Small Anim Pract. 1992;22(5):1145-1153.
- Hayes HM, Wilson GP, Fraumeni JF. Carcinoma of the nasal cavity and paranasal sinuses in dogs: Descriptive epidemiology. Cornell Vet. 1982;72:168-179.
- Reif JS, Bruns C, Lower KS. Cancer of the nasal cavity and paranasal sinuses and exposure to environmental tobacco smoke in pet dogs. Am J Epidemiol. 1998;147(5):488-492.
- Beasley V. Absorption, distribution, metabolism, and elimination: differences among species. In: Beasley V, ed. Veterinary Toxicology. Ithaca, NY: International Veterinary Information Service; 1999. http://www.ivis.org/advances/Beasley/AppC/ivis.pdf?q=venomous-animals-k3. Accessed September 11, 2015.
- Proano M, Camilleri M, Phillips SF, et al. Transit of solids through the human colon: regional quantification in the unprepared bowel. Am J Physiol Gastrointest Liver Physiol. 1990;258(6 pt 1):G856-862.
- Lewis LD, Magerjurth JH, Roudebush P, et al. Stool characteristics, gastrointestinal transit time and nutrient digestibility in dogs fed different fiber sources. J Nutr. 1994;124(12 suppl):2716S-2718S.
- Lorenzo-Figueras M, Merritt AM. Effects of exercise on gastric volume and pH in the proximal portion of the stomach of horses. Am J Vet Res. 2002;63(11):1481-1487.
- Peachey SE, Dawson JM, Harper EJ. Gastrointestinal transit times in young and old cats. Comp Biochem Physiol A Mol Integr Physiol. 2000;126(1):85-90.
- Lewis LD. Feeding and Care of the Horse. 2nd ed. Media, PA: Lippincott Williams & Wilkins; 1996.
- Dresbach DS. Ruminant GI dynamics: effects of pharmacodynamics on oral dosage forms. In: Monkhouse DC, ed. Animal Health Products: Design and Evaluation. Washington, DC: American Pharmaceutical Association; 1978:22-31.
- Beasley DE, Koltz AM, Lambert JE, et al. The evolution of stomach acidity and its relevance to the human microbiome. PLoS ONE. 2015;10(7):e0134116.
- Martinsen TC, Bergh K, Waldrum HL. Gastric juice: a barrier against infectious diseases. Basic Clin Pharmacol Toxicol. 2005;96:94-102.
- Brosey BP, Hill RC, Scott KC. Gastrointestinal volatile fatty acid concentrations and pH in cats. Am J Vet Res. 2000;61(4):359-361.
- Parra R. Comparison of foregut and hindgut fermentation in herbivores. In: Montgomery GG, ed. The Ecology of Arboreal Folivores. Washington, DC: Smithsonian Press; 1978:205-231.
- Riviere JE, Papich MG, eds. Veterinary Pharmacology and Therapeutics. 9th ed. Ames, IA: Wiley-Blackwell; 2009.
- Bonagura JD. Cardiovascular diseases. In: Sherding RG, ed. The Cat: Diseases and Clinical Management. 2nd ed. London, England: Churchill Livingstone; 1994.
- Reece WO, ed. Dukes’ Physiology of Domestic Animals. 13th ed. Ames, Iowa: Wiley-Blackwell; 2015.
- Convenia (cefovecin sodium injectable cats and dogs) Product package insert. Accessed July 27, 2016.
- Raabe BM, Lovaglio J, Grover GS, et al. Pharmacokinetics of cefovecin in cynomolgus macaques (Macaca fascicularis), olive baboons (Papio anubis), and rhesus macaques (Macaca mulatta). J Am Assoc Lab Anim Sci. 2011;50(3):389-395.
- Virginia Tech Institutional Animal Care and Use Committee. Guidelines for regulating the volume of experimental blood sample withdrawals in animals. December 18, 2013. https://ouv.vt.edu/content/dam/ouv_vt_edu/guidelines/guidelines/guidelines-regulating-volume-bloodsamples.pdf. Accessed July 27, 2016.
- Zuber R, Anzenbacherová E, Anzenbacher P. Cytochromes P450 and experimental models of drug metabolism. J Cell Mol Med. 2002;6(2):189-198.
- Archer TM, Boothe DM, Langston VC, et al. Oral cyclosporine treatment in dogs: a review of the literature. J Vet Intern Med. 2014;28(1):120.
- Court MH. Feline drug metabolism and disposition: pharmacokinetic evidence for species differences and molecular mechanisms. Vet Clin North Am Small Anim Pract. 2013;43(5):1039-1054.
- Boothe DM. Small Animal Clinical Pharmacology and Therapeutics. 2nd ed. Philadelphia, PA: Elsevier Health Sciences; 2011.
- Center SA, Elston TH, Rowland PH, et al. Fulminant hepatic failure associated with oral administration of diazepam in 11 cats. J Am Vet Med Assoc. 1996;209(3):618-625.
- Sipes IG, Gandolfi AJ. Biotransformation of toxicants. In: Amdur MO, Doull J, Klaasen CD, eds. Cassarett and Doull’s Toxicology: The Basic Science of Poisons. 4th ed. New York, NY: Pergamon Press; 1991.
- Baggot JD. Principles of Drug Disposition in Domestic Animals: The Basis of Veterinary Clinical Pharmacology. Philadelphia, PA: WB Saunders Company; 1977.
- Thrall MA, Weiser G, Allison R et al, eds. Veterinary Hematology and Clinical Chemistry. 2nd ed. Hoboken, NJ: John Wiley & Sons; 2012.
- Giguère S, Prescott JF, Dowling PM, eds. Antimicrobial Therapy in Veterinary Medicine. 5th ed. Hoboken, NJ: John Wiley & Sons; 2013.
- Kummrow MS, Tseng F, Hesse L. Pharmacokinetics of buprenorphine after single-dose subcutaneous administration in red-eared sliders (Trachemys scripta elegans). J Zoo Wildl Med. 2008;39(4):590-596.
- Holz P, Barker IK, Burger JP, Crawshaw GJ, Conlon PD. The effect of the renal portal system on pharmacokinetic parameters in the red-eared slider (Trachemys scripta elegans). J Zoo Wildl Med. 1997;28(4):386-393.
- Mealey KL, Bentjen SA, Gay JM, et al. Ivermectin sensitivity in Collies is associated with a deletion mutation of the mdr1 gene. Pharmacogenetics. 2001;11(8):727-733.
- Washington State University College of Veterinary Medicine Veterinary Clinical Pharmacology Lab. Affected breeds. http://vcpl.vetmed.wsu.edu/affected-breeds. Accessed July 27, 2016.
- Ramirez CJ, Minch JD, Gay JM, Lahmers SM, Guerra DJ, Haldorson GJ, Schneider T, Mealey KL. Molecular genetic basis for fluoroquinolone-induced retinal degeneration in cats. Pharmacogenet Genomics. 2011;21:66-75.
Back to Top